Introduction
A space program in this new age is constrained by severe safety protocols, precision controls, and maneuverability requirements, and drastic cost reduction mandates. This situation necessitated the development of a low thrust high precision propulsion system as an efficient and cost-effective alternative to the commonly used chemical or advanced chemical systems. Space science explorations and military applications now demand spacecraft, which are characteristically fit for formation flying, attitude and orbit maneuverings, and other high-level capabilities and functions. To these ends, propulsion systems with pulse inductive micro thrusters having smaller thrusts and greater precision appear to be a promising option. This is relatively a new and unconventional line of thinking making way for plasma physics in the field of aeronautics. Plasma propulsion systems have extended the limits of technological advances in the field of space science. Such a system enhances the efficiency and survivability of a space mission and adds to its overall performance characteristics (Eckman, 1999).
Aiming to take stock of the development of different plasma propulsion systems for formation flying, a study on experimental characterization of pulsed inductive micro thrusters has been taken up. This study attempts to investigate the efficacies and impacts of different plasma micro thrusters on overall system performance assessed under the yardsticks of the NASA LISA mission requirements. The findings of this study have been presented in this report. This is the introductory chapter of the study, which provides a brief premiere to the motivation and context of research, research problems, objectives, and methodology of research. The chapter concludes by outlining the structure of the dissertation.
Motivation & Context of Research
The traditional space program taken up for scientific and military applications has come under the scanner mainly because of safety and cost concerns (Ziemer & Merkowitz, 2004). Any space mission now confronts severe challenges as we seek to achieve unprecedented improvements in safety, cost, tenure, precision controls, and other mission performance parameters. This situation witnessed a sudden rise in interest towards formation flying spacecraft for science and military applications. This further necessitated the development of propulsion systems for small spacecraft capable of precision control for multi-satellite guidance functions including attitude and orbit maneuvering (LaPointe, 2001).
Realizing the need for reducing mission costs and augment precision controls, NASA has planned to use formation flying several small and specialized satellites in place of a single complex science platform. The shift in NASA’s approach in favor of multiple small satellites has been motivated by the prospects of lowering satellite production costs, distributing risk across formation elements reducing failures, and enhancing mission precision and flexibility. For near-earth applications, the economy of scale provided by the use of small, low mass satellites allows a single launch vehicle to deliver several satellites to orbit, and low cost piggyback launches on other vehicles can be used to replenish satellite clusters. As noted in a recent publication by the NASA Goddard Space Flight Center, “Formation flying is quickly revolutionizing the way the space community conducts autonomous science missions around the Earth and in space. This technological revolution will provide new, innovative ways for this community to gather scientific information, share this information between space vehicles and the ground, and expedite the human exploration of space.”
Current and near-term NASA scientific missions are testing the limits of formation flying and the positioning accuracy of satellites in orbit as well as to one another. Examples include the Terrestrial Planet Finder (TPF), a series of infrared astronomical telescopes used to detect thermal emissions of planets; Space Technology 5 (ST-5), a precision formation-flying test-bed for innovative technologies to be used on future missions; and the Laser Interferometer Space Antenna (LISA), a space-based gravitational wave observatory. Given the environmentally sensitive nature of the measurements, these missions will require positioning control actuation on exceedingly small scales, for which propulsion systems with smaller thrusts and greater precision than those currently available will be necessary. For instance, to effectively observe gravitational waves, the LISA instrument must operate so that any non-gravitational disturbances such as light pressure or solar wind are eliminated. Therefore, rigid, high-resolution requirements on both the pointing and the translation of the spacecraft need to be implemented. Since the stability of the spacecraft relates directly to the quality of the science measurements, the propulsion system is a critical component. The problem is complex and becomes exceedingly difficult for small spacecraft with extended duration missions when attempting to produce an attitude control system that has a low mass fraction (ratio of propellant mass to overall system mass), which increases system efficiency and maximizes scientific gain. An ideal system would provide a high level of controllability with rapid response times (thrust noise?), and low-thrust levels (i.e., 0.1-30 µN for LISA) with the pulsing capability to ensure precision positioning and guidance accuracy (i.e., resolution <0.1 µN for LISA). Progressive innovations in both system efficiency and mass (reduction) will be essential to meet these needs.
Traditional solutions to propulsion and control systems for interceptor applications have generally utilized some form of chemical or advanced chemical propulsion. Chemical propulsion systems have the advantage of producing large thrusts, but suffer from inefficiency thereby increasing system size. Additionally, increasing chemical system response time and precision impulse bit control to the required levels can be difficult. One possible solution for high-performance control, while providing for the needed reduction in system mass fraction, is the use of a miniature electrostatic or electromagnetic plasma propulsion system. The current generation of electrostatic or electromagnetic micro propulsion options ranges from MEMS-based micro ion thrusters, Field Emission Thrusters (FEEP), and Micro Pulsed Plasma Thruster (PPT). These systems have the potential for attitude control for spacecraft or interceptors with masses of 5 to 10 kg but the propulsion system’s wet masses and power consumption will need to have significant reductions. MEMs based electric thrusters show promise but microgrid erosion and very low thrust levels remain an issue.
Given the potential advantages over the usual large, single-craft platform, many space missions are considering the use of satellite, formation-flying systems to achieve goals that are difficult utilizing standard methods. Often mission objectives are diverse and complex and the array of scientific instruments can present competing and conflicting requirements on a one-satellite design and its operational capabilities. The same complex missions can be achieved by distributing the scientific payload onto smaller, simpler, individual satellites, lowering the effective cost and creating a more flexible, efficient system, while enhancing survivability, and reducing the overall mission risk. Linking the satellites virtually can then provide scientific data previously inaccessible through conventional means.
Research problems & Objectives
The traditional space missions using chemical or advanced chemical propulsion technologies face restrictions concerning cost, flight time, earth orbit-lifting potentials, safety requirements, precision controls, and maneuverability capabilities (See, Ziemba, 2003). Missions like the Voyager-I were based on such conventional chemical propulsion technologies and were thus destined to consume huge financial resources and take substantially longer flight time (Ziemer & Merkowitz, 2004). This is authenticated by the fact that the Voyager-I launched in 1977 reached the boundary of the solar system only now taking a very long time frame spanning so many years. Such extended trip time along with lower payload fractions restrict the maximum scientific gain of traditional space missions. Further, the conventional propulsion system being very expensive and having greater launch weight makes it difficult to take up such missions more frequently (Reichbach, 2001).
Being well aware of the above restrictions and having decided to cut down mission costs and enhance the efficiency and utility of space programs, NASA felt the need for the development of advanced, high precision, and cost-effective technologies for its upcoming missions. Some of the important near-term scientific missions of NASA include ST-3, ST-5, and LISA (Laser Interferometry Space Antenna), a precision formation-flying mission. NASA will launch these missions in the coming years and being technically and functionally more advanced than their conventional counterparts, these missions will require new technologies to produce maximum scientific gains. Such missions will call for position and attitude control actuation on very small scales, for which a low thrust high precision propulsion system is an absolute requirement.
Various propulsion systems are known today, such as field emission electrostatic propulsion thrusters (FEEP), colloid thrusters, pulsed plasma thrusters (PPT), and miniature cold gas thrusters. The capabilities of these space propulsion systems as well as their effects on large-scale space system performance vary between the systems. The selection of a propulsion system may not be easy. The decision of which propulsion system to use for each mission is an important one with both immediate and long-term effects. Not only are mass, power, and maneuvering requirements important for the missions, but the propulsion technologies developed for the near-term missions will greatly influence their use in future missions with similar needs.
Taking note of the above issues, this study seeks to address the problems associated with low thrust high precision space missions and characterize the different propulsion options available for such missions. The study then focuses on analyzing how these options measure up to the requirements of the LISA formation-flying mission and thus identify the most suitable propulsion option. This is the principal aim this study goes on to achieve by exploring and accomplishing a set of milestones or objectives pre-identified for this purpose. The objectives are:
- Review of space mission and propulsion technologies with special focus on high precision formation flying and micro thrusters.
- Investigation of the capabilities of various state-of-the-art space propulsion systems and their overall effects on space system performance.
- Characterization and comparative assessment of different micro pulsed inductive thrusters for low thrust & high precision applications.
- Selection of micro thruster suitable for the NASA-LISA mission scheduled to be launched.
The propulsion options considered for comparative analyses in this study include- FEEP Microthrusters, Colloid Microthrusters, Cold Gas Thrusters, and uPIT Concept. The technical aspects and functional efficacies of these options have been thoroughly scrutinized to identify the salient features of each option from the perspectives of their application as micro thrusters for new-age space missions. Finally, the individual propulsion systems have been assessed against the NASA LISA mission requirements. The LISA mission has been selected as the benchmark as this is one of the advanced and high precision space programs scheduled to be launched in the near term. This mission having the mandates for using state-of-the-art technologies, formation-flying capabilities, and other high precision functionalities appears to be a good candidate for employing advanced inductive propulsion systems thereby justifying the selection of the LISA mission as the benchmark for this study.
Research Approach & Methodology
The primary goal of this investigation is to measure electron temperature and density in the plume of a LeRC PPT operating at energy levels corresponding to the energy range of the proposed LISA mission. This experimental investigation is part of an effort to characterize the PPT plume to assist in the evaluation of potential plume-spacecraft interactions and to develop the ability to predict their impact. The diagnostics used for electron density and temperature measurements in plasmas include single and double Langmuir probes, spectroscopic and Interferometric methods. Single and double probes have the disadvantage of requiring relatively long or reliably repeatable measurements to obtain one data point, while optical and Interferometric methods are hampered by the complexity of the required apparatus (See, Edmund, 2002). In the very short duration (approximately 15 µs) of pulse discharge of the PPT, the triple probe shows great opportunity to easily and reliable measure electron temperature and density. The challenges of using the probes in the PPT plume include the electrically noisy nature of the discharge and the short duration of the pulse, which requires very accurate and sensitive measuring devices.
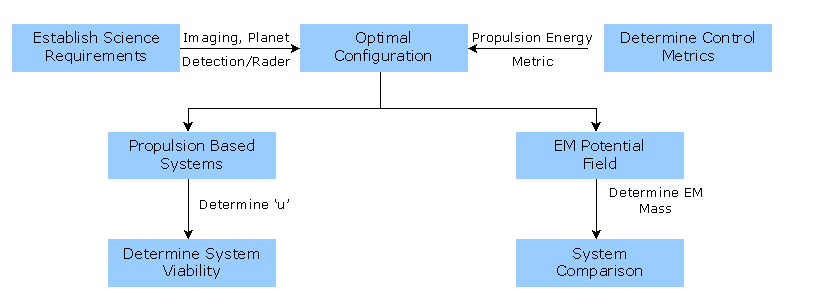
The approaches taken to meet the objectives have been summarized in the flow diagram shown in Figure 1.1. First, the science requirements for the multi-spacecraft system are determined. Together with control metrics, the optimal configuration is then determined. For propellant-based systems, this optimal configuration corresponds to the set of optimal controls required to reconfigure the spacecraft. From these controls, the propellant-related mass can then be determined, thus the overall system mass. The obtained results are then compared to the current system designs to determine the viability of these proposed systems to meet the given science requirements. Further details of the methodology and the experimental setup of this study are included in Chapter III.
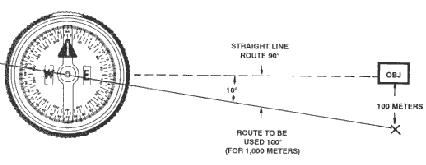
Figure-1.2 outlines the thesis layout. Beginning with this introductory chapter (Chapter-I), this dissertation runs into five chapters in all (Chapter-I to V). Chapter II presents the review of the literature and prior arts about the science of space missions and the development of advanced propulsion technologies
Review of literature
Having identified the research problems and objectives in Chapter-I, this chapter proceeds to review existing literature and prior-arts about space missions and space propulsion technologies. Starting with a brief background on the transformations witnessed in the field of space science and technology, the review highlights the emerging concepts of interferometry and formation flying. An underlying aim of this review is to provide the framework and foundation for carrying out the experimental characterization of pulsed propulsion systems capable of meeting the stringent requirements of the new-age space missions like NASA-LISA, ST-3, SMART-2, etc. proposed to be launched in the coming years. As mentioned earlier, the requirements of the proposed NASA-LISA mission have been considered as the benchmark or criteria for the assessment and selection of propulsion systems in this study. Therefore, it is necessary to include here the details of the LISA mission, its objectives, and the requirements. This chapter is predominantly based on secondary sources of information and special care has been taken to use only authentic or first-hand sources of information like Government reports, technical publications of NASA, research reports of Aerospace Agencies, and other such reliable sources.
Transformations in Space Science
Conventional space programs used relatively bigger and heavier chemical or advanced chemical propulsion systems. Chemical propulsion systems have the advantage of producing large thrusts, but suffer from inefficiency thereby increasing system size (See, Ian, 2000). Additionally, increasing chemical system response time and precision impulse bit control to the required levels can be difficult.
A space program in this new age is constrained by severe safety protocols, precision controls, and maneuverability requirements, and drastic cost reduction mandates (Reichbach et al., 2001). This situation necessitated the development of a low thrust high precision propulsion system as an efficient and cost-effective alternative to the commonly used chemical or advanced chemical systems. Space science explorations and military applications now demand spacecraft, which are characteristically fit for formation flying, attitude and orbit maneuverings, and other high-level capabilities and functions (Gimeno-Fabra, 2001). To these ends, propulsion systems with pulse inductive micro thrusters having smaller thrusts and greater precision appear to be a promising option. This is relatively a new and unconventional line of thinking making way for plasma physics in the field of aeronautics. Plasma propulsion systems have extended the limits of technological advances in the field of space science. Such a system enhances the efficiency and survivability of a space mission and adds to its overall performance characteristics (Eckman, 1999).
The traditional space missions using chemical or advanced chemical propulsion technologies face restrictions concerning cost, flight time, earth orbit-lifting potentials, safety requirements, precision controls, and maneuverability capabilities (See, Ziemba, 2003). Missions like the Voyager-I were based on such conventional chemical propulsion technologies and were thus destined to consume huge financial resources and take substantially longer flight time (Ziemer & Merkowitz, 2004). This is authenticated by the fact that the Voyager-I launched in 1977 reached the boundary of the solar system only now taking a very long time frame spanning so many years. Such extended trip time along with lower payload fractions restrict the maximum scientific gain of traditional space missions. Further, the conventional propulsion system being very expensive and having greater launch weight makes it difficult to take up such missions more frequently (Reichbach, 2001).
Thus, the need for cost-effective solutions allowing for high mass fractions of payload to propellant for in-space propulsion has lead to the development and utilization of plasma-based systems. Plasma thrusters are more efficient than chemical propulsion thus reducing propellant requirements but they suffer from a low thrust (Kirk, 2006).
Space Propulsion Systems
Propulsion systems are the most important components of any spacecraft, which are necessary for the delivery of the payload to its mission location, and for maintaining proper orbital location under the perturbing forces of the Sun and planets (Warner, 2003). Both the launch vehicle used for leaving the Earth’s surface and any onboard propulsion used for orbit insertion and stationkeeping form the propulsion system. Any exploration of the solar system and beyond is not possible without reliable and efficient propulsion systems (Ferguson, 2000).
Propulsion technologies can be of two types in general- chemical propulsion systems and electric propulsion systems. In chemical propulsion, two propellants (fuel and oxidizer) react in a combustion chamber to produce a hot gas, which is expelled through a nozzle as shown in Figure-2.1. Chemical propulsion relies on energy stored within the chemical bonds between atoms or molecules of the propellant and such systems may have performance limitations due to the chemical energy stored in the reaction partners (Tajmar M., 2001). This energy is generally extracted through a combustion or reaction process, where the liberated excess energy produces a high-pressure exhaust and this exhaust is expelled through a nozzle to create thrust for the satellite or the spacecraft.
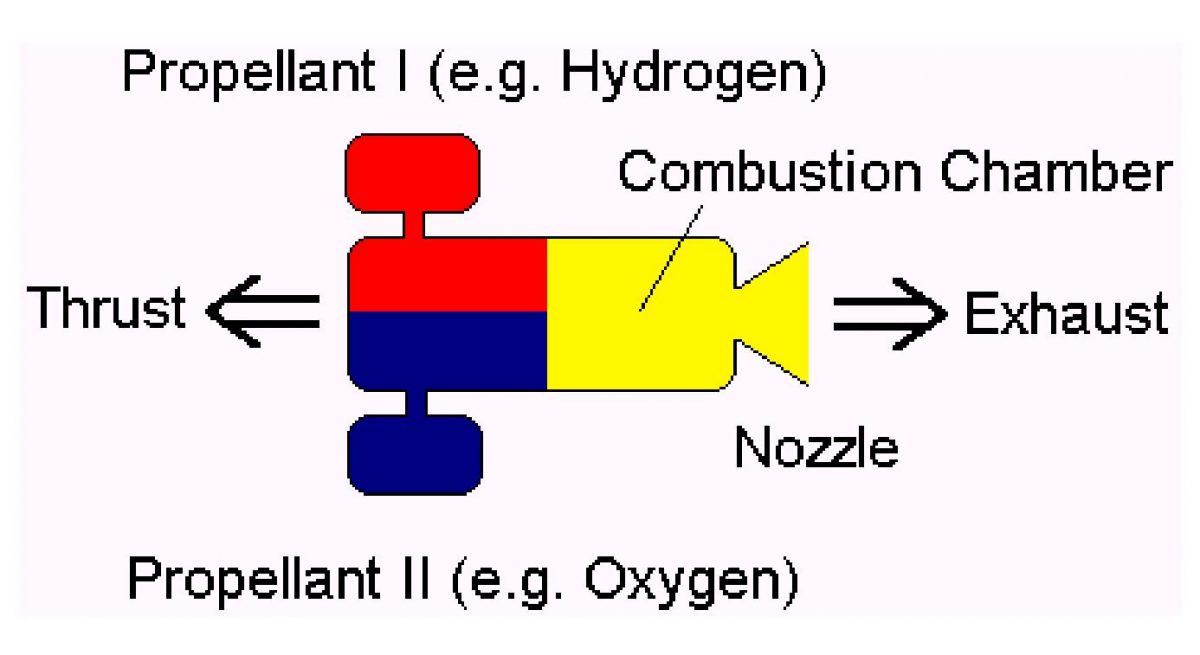
Chemical thrusters can be bipropellants, where the mixing of two reactants starts the reaction and energy liberation process. Smaller chemical thrusters tend to be monopropellant and often have no chemical reaction involved, just utilizing stored energy in the form of tank pressure (See, Yashko & Hastings, 1996). Such thrusters are typically referred to as “cold gas thrusters.”
There exists another type of chemical thruster where a solid propellant is burned to produce a high-pressure exhaust and these are commonly known as Solid rocket motors. The most efficient chemical engine built to date is the Space Shuttle Main Engine (SSME), which has a specific impulse of 465s in a vacuum (Tajmar M., 2001). Chemical thrusters typically have specific impulses on the order of several hundred seconds.
Another category of advanced propulsion systems exists that uses energy generated onboard the satellite (solar arrays, nuclear power source) to couple with a working fluid or gas. Depending on the power available, performance and propellant utilization can be drastically increased in this case. These are electric thrusters and unlike chemical thrusters, electric thrusters can operate at much higher specific impulses (Ian, 2000). They rely on an external power supply to provide input power that is utilized in a variety of different ways, depending on the type of thruster.
Electric thrusters typically come in three types: electrothermal, electrostatic, and electromagnetic. Electrothermal thrusters operate similarly to chemical thrusters, except a heater is used to additionally increase the temperature of the propellant, thereby increasing the efficiency of the thruster. Electrostatic thrusters typically use a plasma discharge (Ion and Hall thrusters) or some sort of liquid propellant that can be easily ionized (Colloids, FEEPs). Thrust is produced by accelerating ions through electric fields. The specific impulse of electrostatic thrusters is only limited by the input power used to accelerate the ions. As more power is provided, the ions can be accelerated to greater velocities and specific impulse is increased. This theory has no upper limit (except the speed of light).
Electromagnetic thrusters utilize both electric and magnetic fields to accelerate charged particles and produce thrust. These thrusters typically operate with a plasma discharge (MPD thrusters). Electromagnetic thrusters can produce relatively high thrust for an electric propulsion device, but their specific impulses are more limited than electrostatic thrusters.
These two main divisions of in-space propulsion technology represent two different operational purposes. Chemical propulsion is typically used for high-thrust maneuvers and has low fuel efficiency compared to electric propulsion. Launch vehicles use chemical propulsion systems because they produce the high thrust necessary for Earth escape. Electric propulsion is aimed more at low-thrust missions such as stationkeeping and orbit transfers where time to transfer is not the highest priority. Many forms of electric propulsion require a low-density vacuum environment to operate and are thus precluded from launch vehicle use, where operations typically begin near sea level. The benefit of electric propulsion is seen in its fuel efficiency, which is often several times that of chemical rockets.
As the onboard availability of power increases for spacecraft in the future, likely through nuclear power technologies, higher power electric propulsion will become possible and the large gap in thrust between electric and chemical propulsion technologies will begin to close.
References
Ziemer K. John and Merkowitz M. Stephen, “Microthrust Propulsion for the LISA Mission”, California Institute of Technology/ NASA Goddard Space Flight Center, Paper presented at the 40th AIAA/ASME/SAE/ASEE Joint Propulsion Conference and Exhibit, Fort Lauderdale, Florida, 2004, pp. 1-2.
LaPointe R. Michael, “Formation Flying with Shepherd Satellites”, Ohio Aerospace Institute, Cleveland, OH 44142, Phase-I Final Report, 2001.
Ziemba M. Timothy, “Experimental Investigation of the Mini-Magnetospheric Plasma Propulsion Prototype”, University of Washington, 2003.
Reichbach Jeffrey, “Micropropulsion System Selection for Precision Formation Flying Satellites”, Massachusetts Institute of Technology, 2001.
Eckman F. Robert, “Langmuir Probe Measurements In The Plume Of A Pulsed Plasma Thruster”, Worchester Polytechnic Institute, 1999.
Edmund Mun-Choong Kong, ‘The Spacecraft Formation Flight-Exploiting Potential Fields’ University of Queensland, Massachusetts Institute of Technology, 2002.
Reichbach G. Jeffrey, Sedwick J. Raymond, and Martinez-Sanchez Manuel, “Micropropulsion System Selection for Precision Formation Flying Satellites”, 2001 SERC #1-01.
Gimeno-Fabra Lluís, “Design, Manufacture, and Properties of Cr-Re Alloys for Application in Satellite Thrusters” European Aeronautic Defence and Space Company, EADS Corporate Research Center, Germany. Department of Metallic Structures, 2001.
Ian J. E. Jordan, “Electric Propulsion: Which One For My Spacecraft”? Whiting School of Engineering, 2000.
Kirk D. R., “Introduction to Electric Propulsion”, Mechanical and Aerospace Engineering Department, Florida Institute of Technology, 2006.
Warner Z. Noah, “Performance Testing and Internal Probe Measurements of a High Specific Impulse Hall Thruster”, Aeronautics and Astronautics, Massachusetts Institute of Technology, 2003.
Ferguson A Philip, “Distributed Estimation and Control Technologies for Formation Flying Spacecraft”, Aerospace Engineering, University of Toronto, 2000.
Tajmar, M., “Future Challenges in Spacecraft Plasma Interactions: Plasma Propulsion and Gas Release”, Austrian Research Centers, Seibersdorf, A-2444 Seibersdorf, Austria, 2001.
Yashko G.J. and Hastings D.E., “Analysis Of Thruster Requirements And Capabilities For Local Satellite Clusters”, Massachusetts Institute of Technology, Cambridge, Massachusetts, 1996.