Abstract
Historically, earthquakes have caused immense human and property loss. Besides the valuable human lives lost, earthquakes have caused monetary losses rising to billions of dollars. In the wake of these disasters, engineers have tried to design and construct earthquake-resistant buildings. These buildings adopt new technologies and processes in detecting and reducing earthquake forces. This paper identifies the base isolation systems, reinforcement of building materials, proper choice of building materials, and effective formulation and implementation of safety guidelines as the main pillars underlying the construction of earthquake-resistant buildings. The adoption of earthquake-resistant technology in a seismic area supports the adoption of this technique. The measures identified in this study, therefore, work to mitigate the impact of an earthquake when it occurs. Comprehensively, this study recommends more research to identify preventive measures that mitigate the impact of earthquakes on buildings (and especially on the people that reside in them). Such measures may involve the installation of seismic activity detectors and similar initiatives. The findings of this paper are therefore useful in improving the ability of buildings to withstand future seismic forces.
Introduction
Earthquake-related deaths occur because buildings collapse in the wake of strong earth movements. Unfortunately, after spending decades in the advancement of engineering technology, minimal results exist to prevent death and damages caused by the collapse of buildings. The inadequacies in the production of effective earthquake-resistant construction methods show a weakness of engineering technology. Historically, earthquakes have caused widespread human devastation. For example, in 1908, the Messinia earthquake in Italy caused a human death toll of about 100,000 (International Association for Earthquake Engineering 2002). In addition, between 2010 and 2011, earthquakes have shaken most parts of America, Asia, Europe, and South America (such as the 2010 8.8 magnitude earthquake in Chile) (Cardenas 2012). In 2011, a 5.8 magnitude earthquake also hit the Atlantic seaboard and its surroundings (this earthquake also damaged the Washington monument) (Ford 2012).
Even though the above statistics paint an ominous future, it is vital to point out the predominance of earthquakes in natural events. It is almost impossible to prevent earthquakes but the International Association for Earthquake Engineering (2002) says the human resilience to survive them has been unending. Through this observation, one thing has come out clearly; earthquakes (in their nature) do not kill people, because the structures we build around us do (International Association for Earthquake Engineering 2002). This statement may however sound general and oversimplified because the aftermaths of earthquakes (like tsunamis) also kill people. Even though not all tsunamis are a product of earthquakes, they are a primary catalyst for the weakening of buildings because structures exposed to severe environmental catastrophes such as tsunamis experience sudden lateral accelerations. One logical question arises in this observation – is it possible to construct buildings or structures that withstand these devastating natural events?
The answer to the above question lies in the conviction of engineers who deem it possible to construct earthquake-proof buildings that are able to withstand even the most severe earthquakes. Some engineers even provide the surety that earthquake-proof buildings can look as good as new once the shaking ends (International Association for Earthquake Engineering 2002). Nonetheless, at this point, it is crucial to point out that constructing an earthquake-proof building is very costly and therefore, many developers have resorted to pursuing a more practical option, which is to construct earthquake-resistant buildings. Unlike earthquake-proof buildings, earthquake-resistant buildings only prevent the total collapse of buildings to prevent death if a disaster occurs.
Recently, there has been tremendous progress made in earthquake engineering. The field of earthquake engineering is not new. Proof of this fact stems from the resilience of old buildings in surviving earthquakes (despite their close proximity to highly active seismic zones). One such building is the Hagia Sophia building in Turkey (which recently converted to a museum). The building’s construction started and ended in 537 A.D but it still stands today. There was a time in its history when a massive earthquake affected the building (two decades after its construction) but after its reconstruction and reinforcement, the building has been able to withstand most natural disasters (Denny 2010, p. 135). Comprehensively, the International Association for Earthquake Engineering (2002) says the techniques for making earthquake-resistant buildings have changed but the main principles underlying its formulation remain the same.
This paper also affirms this fact by demonstrating that new techniques for building earthquake-resistant technologies stem from common principles in earthquake engineering. These principles work to reduce seismic forces that destabilize buildings. To explain the existing and potential techniques for constructing earthquake-resistant buildings, this paper emphasizes the importance of understanding seismic forces, their capability, and how to mitigate the destructive nature of earthquakes occur.
Comprehensively, this paper consists of four chapters. The first chapter introduces this paper, while the second chapter explores the nature of earthquakes, the impact of earthquakes, and the reason buildings fail to withstand earthquakes. Through a case study approach, this chapter also explores why buildings have historically failed to survive earthquakes. Lastly, this chapter explains the advantages and benefits enjoyed from adopting earthquake-proofing techniques and the theoretical background informing their adoption. The third chapter examines the intricacies involved in building earthquake-resistant buildings, the methodologies involved, and the application of these techniques in real-world settings. Finally, the last chapter outlines the conclusion and recommendations of this paper.
Literature Review
Nature of Earthquakes
To understand the impact of earthquakes on buildings, it is important to understand the definition of earthquakes. Gupta (2011) says earthquakes are sudden movements of the earth’s crust, which destabilize the earth underneath through seismic waves. Earthquakes do not happen in a definite place because they can occur on the ocean floor or even on dry land. However, cities that stand along fault lines (or highly volcanic areas) experience the highest frequency of earthquakes (this fact does not mean other places cannot experience earthquakes) (International Association for Earthquake Engineering 2002).
From the human and structural devastation of earthquakes, there has been a global clamor to construct buildings in a quakeproof way. Nonetheless, the surety that at least some level of precaution insulates buildings against earthquakes cannot guarantee the full protection of buildings against earthquakes. So far, scientists have invested many resources to explore better ways to protect buildings against earthquakes. Consequently, many engineers develop building technologies to protect buildings against the severe effects of earthquakes but still the International Association for Earthquake Engineering (2002) says none of these technologies provide 100% protection against earthquakes.
Impact of Earthquakes on Buildings
As demonstrated through the widespread human and capital loss experienced from earthquakes, there is a profound impact of earthquakes on buildings. The impacts of earthquakes on buildings occur because the movement on the earth’s crust propagates seismic energy that shakes the earth, causing the ground to shake (Storgaard 2010). This sudden motion causes buildings to shake and eventually collapse. Seismic waves that cause these motions are often classified into two groups: body (P and S waves which travel through the earth’s interior) and surface waves. To explain the P and S waves, Storgaard (2010) says,
“P waves resemble sound waves, which mean they compress and expand the material as they pass. S waves resemble water waves, which means they move material up and down. P-waves travel through both solids and liquids, while S waves only travel through solids” (p. 9).
In an earthquake, P-waves are the first to occur, and later, S-waves release (afterward, surface waves occur) (Storgaard 2010). Geologists sometimes refer to surface waves as Rayleigh waves. Other geologists refer to this wave as love waves (Storgaard 2010). Both waves cause major damage to the earth’s surface because they have the potential to move the ground horizontally and vertically (however, only Rayleigh waves have the potential to move the ground vertically).
Vertical movements of the earth’s surface may not cause a lot of damage to the earth because engineers design buildings to withstand vertical forces (like forces of gravity). Horizontal forces are therefore the main causes of damage to buildings. Love waves are the most lethal horizontal forces in an earthquake because they are very strong and can easily cause a building to collapse. These forces similarly cause lateral accelerations used by scientists to measure G-forces. While explaining this phenomenon, Storgaard (2010) said, “An earthquake of a 6.7 magnitude, for example, can produce an acceleration of one G and a maximum velocity of 40 inches (102 centimeters) per second” (p. 13). This force may affect any building because when explained differently, it is like someone shoving another person violently. A building’s structural elements are therefore likely to weaken under such extreme forces. In fact, beams, columns, walls, and floors are likely to crack or dissipate under such a force (thereby leading to the complete collapse of a building).
Another risk factor that manifests in earthquakes is liquefaction. Liquefaction usually leads to the collapse of buildings because the process softens a building’s foundation to become fluid (thereby causing the complete collapse of a building) (National Research Council (U.S.) 1985). Buildings that have firm foundations do not however suffer from liquefaction. This risk forces many engineers to consider the site location for their prospective buildings. Comprehensively, earthquakes weaken buildings and make them vulnerable to collapse.
Why Buildings Collapse in Earthquakes
Seismic forces create shocks that move in ripples to destabilize buildings. Most buildings are naturally able to shoulder their weight but those that destabilize under the impact of an earthquake collapse under their weight. Normally, buildings can carry their own weight and even extra weight from people or even snow. Therefore, from this understanding, engineers expect buildings to sustain an “up and down” movement. Side-by-side movements, therefore, pose the greatest threat to the stability of buildings. Only buildings that withstand these side forces (in the design stage) may also withstand rapid side movements (National Research Council (U.S.) 1985).
Unfortunately, the vulnerability of buildings to earthquakes can only be established when an earthquake strikes (this is a wrong time to do so because lives may be lost and many destructions to property may occur) (International Association for Earthquake Engineering 2002). Very weak buildings may even crumble when they experience just a little force from the ground. Seismic waves are the most lethal force (to buildings) because they strengthen with every vibration (International Association for Earthquake Engineering 2002). Therefore, even the strongest buildings can vibrate like a huge tuning fork when they experience such seismic waves (International Association for Earthquake Engineering 2002). Buildings that have a weak foundation also suffer a high probability of collapsing (Mexico is one such place where buildings have weak foundations).
A building’s vulnerability to earthquake forces manifests through the weight exerted on its foundation. With the addition of every floor, a building acquires more weight and becomes more vulnerable to earthquakes. Therefore, tall buildings are more vulnerable to earthquakes because they exert more weight on their foundations. Tall buildings, therefore, require very strong foundations that withstand the force of an earthquake (International Association for Earthquake Engineering 2002). However, many buildings are susceptible to earthquakes because, while additional weight is increased, the building’s foundation remains the same.
A building’s main strength (when an earthquake occurs) stems from its partition and walls. However, when there is a strong earthquake, these partitions weaken. Therefore, a building becomes weaker with the collapse of the walls (International Association for Earthquake Engineering 2002). Consequently, even a small aftershock wave (in a slightly different direction) would cause the building to collapse. For many buildings, the strength of the wall holds the floor and roof, therefore, when the walls weaken, the floor and the roof eventually collapse. In addition, many buildings have excess weight on their tops (probably because of excess weight from stones to prevent the roof from blowing off, or the installation of water tanks and similar weights on the rooftops). These weights often led to the realization of small cracks on the wall and an outward budge of the reinforcing walls. Eventually, the main steel bars (that hold the building together) weaken by bending outwards. Finally, the structural makeup of the building completely weakens, thereby making the entire building vulnerable to earthquakes. The collapse of the Oakland freeway (in California) demonstrates this weakness because the weakening of the concrete columns caused its collapse. To repair it, engineers had to introduce massive reinforcement belts to the building’s structural dynamics to make it stronger.
For most buildings, the lower floors have more space because of expanded headroom and more room space. These spaces mean lower floors have taller columns and fewer walls. Therefore, lower floors usually carry more weight and bear the highest loads from seismic forces. The International Association for Earthquake Engineering (2002) says, this way, lower floors have the least end fixity and are the first to crumble under the might of an earthquake (the collapse of only one lower floor would lead to extreme disaster). This eventuality manifested in several countries including “Armenia, Mexico, Turkey, Iran, Peru, and now Pakistan and Kashmir” (International Association for Earthquake Engineering 2002, p. 5).
Failure of Engineering Methods in Past Earthquakes
Loma Prieta Earthquake
The Loma Prieta earthquake (in California) occurred in 1989, causing severe damages to buildings and the loss of human life. The earthquake killed more than 70 people and injured 3,500 others (O’Rourke 1992). More people lost their homes after the earthquake-ravaged through major districts and counties in San Francisco. The earthquake measured 6.9 on the moment magnitude scale (O’Rourke 1992).
After reviewing the damage caused by the earthquake, observers reported new engineering failures. One such failure was the lack of supportive sheer walls on the building’s lower floors to prevent it from completely collapsing (International Association for Earthquake Engineering 2002). As observed from the image below, the rough board siding of this building collapsed from the stud wall. Therefore, only the support from the extreme ends of the building prevented its complete collapse
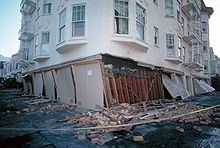
The Loma Prieta earthquake also exposed the dangers posed by the excessive pounding of buildings against one another (this event caused at least one death). O’Rourke (1992) says, assessing the lateral displacement of each building can prevent buildings from pouncing against one another.
The 1964 Japan Earthquake
The 1964 Niigata Japan earthquake measured 1.6 on the Richter scale (National Research Council (U.S.) 1985, p. 32). Its epicenter was located on the Northwest Coast of the country and its impact caused the massive collapse and destruction of buildings in the Northern city of Niigata. More than 3,500 houses completely collapsed, while thousands more suffered significant damage (National Research Council (U.S.) 1985). Most of the collapsed buildings suffered from the effect of liquefaction. Soil liquefaction also caused several sand compaction and volcanoes, while subsidence of up to 140 centimeters occurred in most of the affected areas (National Research Council (U.S.) 1985). In one street containing several apartments, the apartment blocks inclined towards one side. In fact, the diagram below shows one building almost completely overturned because of loose soil.
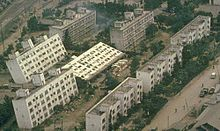
This incidence happened although seismic acceleration sensors (placed on each building) recorded relatively low levels of ground acceleration.
Another significant damage from the earthquake occurred after the collapse of the Showa Bridge. Observers say the damage occurred 70 seconds after the earthquake (National Research Council (U.S.) 1985). Here, liquefaction also manifested as the main cause of the earthquake and not the ground acceleration forces. The earthquake, therefore, highlighted the importance of strengthening a building’s foundation as a step towards making the building more earthquake-resistant (National Research Council (U.S.) 1985).
Sichuan Earthquake
The 2008 Sichuan earthquake occurred in China leaving a fatality of more than 68,000 people (Macdougall 2011, p. 115). This earthquake was massive because it measured 8.0 on the Richter scale. The impact of this earthquake was felt in far-flung cities such as Beijing and Shanghai because distant buildings shook and cracked from the force of the earthquake. Aftershocks from the earthquake also occurred more than five months after the earthquake, thereby increasing the damages and fatalities. The Chinese government declared that it would spend more than $120 billion to rebuild the damaged infrastructure (Macdougall 2011).
The main lesson learned from the Sichuan earthquake was the importance of assessing the impact of the topography of the land before constructing new infrastructures. This importance manifested from the fact that most of the damages reported from the earthquake occurred from landslides and rocks falling. Gravity was the primary force that caused the landslides and rockfalls but according to a preliminary assessment of the Sichuan earthquake, the stability of the land also contributed to the devastating impact of the earthquake (Macdougall 2011).
Northridge Earthquake
The Northridge earthquake occurred in California, but one neighborhood significantly experienced its impact. Even though the earthquake only measured 6.7, its ground acceleration force was among the highest in a California urban neighborhood (Schiff 1995). About 57 people died in this earthquake and more than 8,000 people were injured (Schiff 1995). This earthquake also stood to be among the costliest earthquakes because its cumulative damage was $20 billion (Schiff 1995). The Northridge earthquake highlighted several weaknesses in the construction of most buildings. For example, the earthquake destroyed the joints of the Kaiser Permanente building (which led to its collapse). The end shear walls of the building also collapsed after the building’s lateral load tore it apart. This damage occurred because of the inadequate through-ties holding the building’s lateral load (Schiff 1995).
Whittier Narrows Earthquake
The Whittier Narrows Earthquake measured 5.4 on the Richter scale (Rouhani 1996, p. 244). Its strongest intensity occurred in San Gabriel Valley (in California) and its epicenter was at Rosemead town. The earthquake caused more than $350 million in damages and significantly affected about 10,000 buildings (Rouhani 1996). From the total damages imposed on the buildings, three buildings completely collapsed, while several more suffered irreparable damages (some countries declared a state of emergency so that the affected building owners could receive financial assistance).
Seismic engineers said most of the damages realized from the building occurred because of the “sliding off foundation” effect. For example, the Garvey West Apartment building is a superstructure in the earthquake-hit area and it shifted more than ten inches to the right (Rouhani 1996). Preliminary investigations on the building’s performance showed that the failure to reinforce the building’s foundation was a primary cause of the foundation shift. Therefore, the building was vulnerable because of the failure to incorporate the base isolation technique (International Association for Earthquake Engineering 2002). These failures highlight the importance of pre-empting damages to buildings.
The 2011 Japan Earthquake
The recent Japan 8.9 magnitude caused a Tsunami that killed thousands of people and left many more homeless. This earthquake also caused massive destruction of property and a nuclear disaster in its wake. Even though Japanese officials claimed no significant radiation leakages, the government advised its people to move away from the affected nuclear power plants. Dozens of cities and villages experienced the impact of the tsunami as reports showed that far-flung cities such as Tokyo (which was hundreds of miles away) felt the impact of the earthquake and the triggered tsunami. More than 50 aftershocks occurred after the earthquake and buildings burned down as cars and houses drifted inland (Amidon 2011). Even for a country that had experienced several earthquakes, the 2011 Japanese earthquake disaster was significantly devastating.
Amidon (2011) says that if any person bothers to look at the pictures and the images that characterized the Japan disaster, it is almost inevitable to ask if the country is prepared to face a similar catastrophe again. Nevertheless, many pieces of evidence show that mistakes still occur in the design and construction phases of new buildings that come up today. For example, greedy developers (or rogue developers) show little consideration to the effect of earthquakes on such buildings, and therefore, they develop poor building designs. Perhaps competition and other aspects of the market forces drive many developers to produce mediocre building designs, but undoubtedly, such buildings are of poor quality. Some developers also produce inferior building designs so that they can sell quickly and make a handsome profit. In such cases, there is little or no regard for the quality of the buildings because designers only concentrate on the commercial aspects of the building at the expense of safety aspects. Since such buildings offer very little resistance to earthquakes, Singh (1999) encourages authorities to take up the challenge and enforce high safety standards. Such safety standards should resemble monumental buildings that depend on high precision. Unlike commercial buildings, these monumental buildings have a historic or cultural value that future generations can identify with. Singh (1999) also recommends that it is time for developers who construct commercial buildings to inculcate certain sustainable features of developing monumental buildings into their designs.
Positive Effects of Adopting earthquake-proofing Measures
Introducing earthquake-proofing measures poses several benefits to the protection of human life and property. Some of these methods are inexpensive because they involve cost-effective measures, which require minimal investments. Significant benefits have been realized in several cities and countries (around the world) that have embraced earthquake-proofing measures
For example, by incorporating earthquake-proofing measures in Italy’s construction industry, the country’s vulnerability to earthquakes was reduced. Comparatively, a few years after the 1908 Italian earthquake, a lower death toll occurred in San Francisco after employing earthquake-proofing measures. The low death toll occurred because of using wood as the main construction material. Therefore, only a small death toll of 700 people occurred (despite the fact that this earthquake was bigger than Italy’s). Similarly, observers reported that the survival rates for the San Francisco earthquake were as high as 98% while in Italy, people only had a 30% rate of survival (International Association for Earthquake Engineering 2002). Contractors can therefore not overlook the importance of sound building practices because even a small rupture in the structure of a building can cause thousands of deaths.
Theoretical Background
Reid’s Elastic Rebound Theory
After the 1906 California earthquake, Agarwal (2006) says there was a 250 miles fault line detected to explain the earthquake. On one side of the fault line, the earth slipped more than 20 feet (compared to the other side of the fault line). The emergence of this fault line attracted many scientists (although nobody properly understood what caused earthquakes then). According to the displacement witnessed from the earthquake, a professor of geology at the John Hopkins University said, the earthquake must have occurred from an “elastic rebound” of stress that had been previously stored in the earth (Agarwal 2006). This process informs the concepts surrounding Reid’s elastic rebound theory because the theory stems from the accumulation and eventual release of stress (Agarwal 2006, p. 3).
To demonstrate the elastic rebound theory, Agarwal (2006) recommends envisioning two forces acting on a block of unstrained existing fault. Furthermore, Agarwal (2006) recommends envisioning one force moving a block of land northwards and the second force moving the other block southwards. Initially, there is bound to be no movement because of strong friction between the two blocks. However, with the persistence of this force, fault lines form. Once the force persists for long, Agarwal (2006) says that the weakest point of the fault ruptures and tears (the tear extends rapidly across the fault line). The blocks, therefore, move to a less strained position through the elastic rebound process that generates seismic waves. Heat and other forms of seismic waves manifest through this process. According to Agarwal (2006) earthquakes occur in this manner.
Theory of Plate Tectonics
The theory of plate tectonics works on the premise that earthquake epicenters distribute unevenly across the surface of the earth. This theory investigates why a narrow zone of seismic activity occurs in specific zones (and why volcanoes and other mountainous regions characterize these zones). In fact, Agarwal (2006) explains that 99% of the earth’s most active seismic zones are located within these narrow zones of interpolating seismic activity. Only about 1% of the earth’s global seismicity is away from the narrow and active seismic zones. The rest of the earth is therefore free from these aggressive seismic forces (however, no region of the earth is completely free from earthquakes) (Agarwal 2006). The theory of plate tectonics has gained prominence in the last few decades because it tries to explain how seismic activities occur and why it distributes unevenly in specific regions of the earth.
Alfred Wegener (a German meteorologist) first developed the theory of plate tectonics from the continental drift theory, which explains the origin of continents. Alfred Wegener proposed that all continents formed one block and later, they split to hold their present positions on the earth’s surface. This theory however failed to gain acceptance within the scientific field because Wegener was unable to explain the nature of the force that was strong enough to pull apart the large masses of land (continents) (Agarwal 2006). Another criticism advanced by Harold Jeffrey (a renowned English geophysicist) claimed that it was impossible for rocks that form the bedrock of continents to move that far apart into the ocean without disintegrating. Wegner died in 1930 and before the 1950s, scientists perceived his continental drift theory as improbable and preposterous (Agarwal 2006). After 1950, new debates started to emerge regarding Wegner’s provocative ideas (after new shreds of evidence leaned towards justifying Wegner’s continental drift theory).
One such evidence was the demonstration of ocean ruggedness and the youth of the ocean floor (which supported the view of continents drifting apart). The reversal of the earth’s magnetic field plus the emergence of the seafloor-spreading hypothesis also supported the continental drift theory. Finally, the availability of scientific evidence to show that the earth’s highest concentration of earthquake activities happened on oceanic trenches also reinforced the continental drift theory (Agarwal 2006).
Building earthquake-resistant Buildings
Mapping Seismic Zones
Whenever building contractors are required to undertake any construction process, they are normally attentive to seismic zones. Ideally, this is the first step to developing earthquake-resistant buildings. Many countries have a common institution that maps out the main seismic zones. In Australia, the Australian National Seismic center undertakes this role, while the U.S Geological survey maps the National Seismic Hazardous areas (Harris 2012). These institutions strive to predict ground motions within long periods (say, 50 years). To do so, scientists usually consider historical data and provide an educated guess of the ground forces that may occur within and around the seismic zones.
To formulate perfect seismic design provisions, developers merge international building codes with recommendations from geology institutions. The stakes in building along seismic areas are high because public facilities like bridges and highways need to withstand strong seismic forces from earthquakes. Conversely, in low-risk areas, engineers do not experience the trouble of engaging in complex design processes to increase a building’s resistance against earthquakes. Therefore, the determination of the right seismic activity of a proposed site is an important process in the design of earthquake-resistant buildings. Ideally, buildings that have L or T structures withstand earthquakes, but asymmetric building designs are discouraged because of their vulnerability to earthquakes (International Association for Earthquake Engineering 2002).
Making buildings symmetric is however only the first step of designing buildings to be earthquake-resistant. earthquake-resistant buildings should also withstand the strongest earthquakes (lateral forces). Harris (2012) demonstrates the ability of engineers to counter lateral forces in two ways – improving horizontal and vertical structural systems. This way, engineers ensure the floor and the roof of the buildings are properly reinforced (engineers place a diaphragm on every deck). To reinforce the horizontal structure system, diaphragms are used (the design of Diaphragms mainly enables the sharing of horizontal and vertical forces). The roof however poses a challenge to the installation of diaphragms because it is difficult to place diaphragms here. However, by placing trusses in the rectangular areas of the frame, the problem disappears.
Regarding the vertical structure system, an engineer’s main role is to ensure the building’s columns, beams and braces transfer lateral forces down to the ground (several options can however be explored when building vertical systems). In an earthquake, sideways motions are common. In vertical systems, walls are constructed using braced frames (after including trusses) to counteract sideways forces. Cross bracing (the use of two diagonal trusses) usually offers better and stronger resistance to sideways motions. Moreover, Harris (2012) also explains that
“Instead of braced frames or beside them, engineers may use shear walls – vertical walls that stiffen the structural frame of a building and help resist rocking forces. Engineers often place them on walls with no openings, such as those around elevator shafts or stairwells.” (p. 4).
Nonetheless, when engineers use sheer walls, there is a limit to the dynamics of the building’s design (moment resisting frames are however used occasionally to overcome this limitation). When developers use this design, columns and beams can bend but the joints that hold them together remain firm. “As a result, the whole frame moves in response to a lateral force and yet provides an edifice that is less obstructed internally than shear-wall structures” (Harris 2012, p. 4). From this flexibility, it is easier for designers to incorporate architectural elements such as exterior walls and partitions. Similarly, designers have the flexibility to include loose types of equipment or attractive furniture.
Conventional Seismic Reinforcing
Conventional seismic reinforcing methods include the addition of new materials and the enlargement of existing materials to increase a building’s resistance to earthquakes. The addition of shear walls and bracing is one technique commonly used in conventional building techniques. This technique is the most common technique used in earthquake engineering because it has a relatively cheaper cost of installation, is effective, and it is relatively easy to install. Clearly, the post-cast sheer walls and steel braced frames stand out as the most effective ways for constructing earthquake-resistant buildings. The steel braced frame is more effective in this regard because it has a higher ductility. However, the post-cast concrete method is more common because construction experts are more familiar with it (the addition of sheer walls normally increases a building’s resistance to lateral forces). This way, the load demand on the beams and columns significantly reduces and the building fully reinforces (Harris 2012). The beams and columns (which may still show some weakness after this reinforcement) could be further reinforced through concrete or steel jacketing. These techniques are relatively more laborious. The main aim of undertaking this reinforcement procedure is to optimize a building’s retrofit design so that satisfactory structural performance is achieved using minimum cost.
Use of Modern Innovative Materials
Many engineering methods that strive to improve building materials for earthquake resistance stem from the reinforcement of concrete or steel (few techniques work by reinforcing plastic composites). The use of reinforced plastic has become a very common procedure in developing earthquake-resistant materials. The last decade has especially seen the biggest increase in the use of reinforced plastic (Harris 2012). Stiffness, environmental safety, and durability are some of the reasons developers have adopted reinforced plastics around the world. These advantages have made reinforced plastic composites to be a very competitive alternative to conventional strengthening methods. In addition, reinforcing plastics can strengthen columns, beams, and slabs to make traditionally constructed buildings last longer.
Adobe Construction
Reinforcing building materials can occur from using ductile materials such as bamboo, reeds, or cane so that the wall ties to the foundation (to restrain out-of-plane bending). The reinforcements should transfer bending or inertia forces to transverse walls so that they withstand strong seismic forces. This way, it is easier for the building to withstand vertical crack forces or the shear stresses that may weaken adjoining walls. Using nylon strands, horizontal and vertical reinforcements should tie to the foundations, ring beam, and similar structural elements that support the building (Blondet 2003). The product is a strong matrix that improves the building’s stability if an earthquake occurs. However, Blondet (2003) cautions that engineers should place these reinforcements over every building block as outlined below
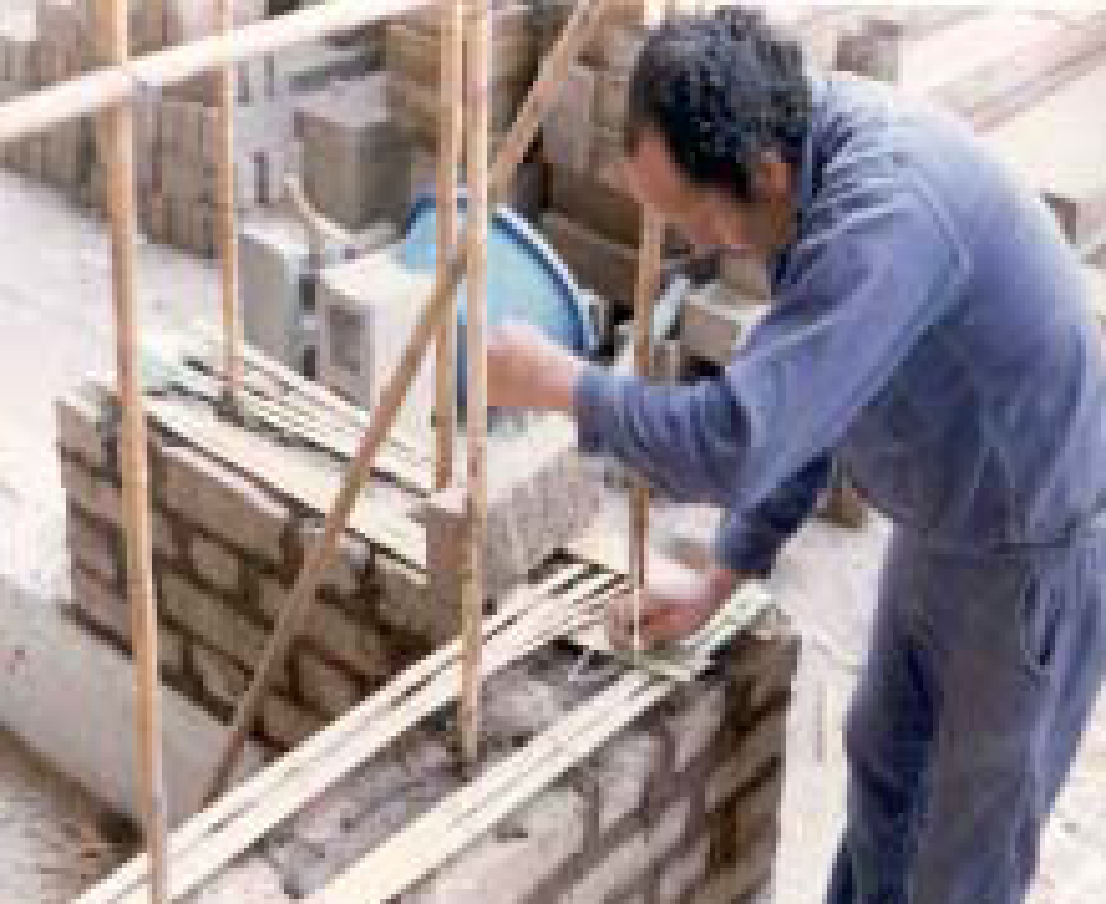
Several structures around the world have used the above technique (including the Catholic building in Peru) (Blondet 2003). Several tests informed this technology including the use of bamboos and other materials over every fourth block. These reinforcements are designed in a manner that the vertical and horizontal reinforcements meet to prevent the separation of walls (at the corners) whenever an earthquake occurs. The structural integrity of the building also stands strong even after an earthquake affects the building. Two experimental tests confirmed this integrity. In one experiment, a building’s corner walls were unreinforced while on the other building, the corners were reinforced using bamboo. A shaking table shook the two buildings (the size of a room) and the unreinforced building suffered severe cracks on the wall, while the reinforced building still stood strong (Blondet 2003). These experiments showed the ability of reinforced walls to withstand earthquakes.
Buttresses and Pilasters
The use of buttresses and pilasters is also another common way of reinforcing building walls. Buttresses and pilasters work by increasing a building’s stability and stress resistance to prevent inward or outward overturning (of the walls). The use of buttresses and pilasters also improves how building blocks interlock so that they are firmer and stronger. Buttresses usually take the form of perpendicular bracing walls, while pilasters take the form of overlapped crossed-over walls. El Salvador has been a leader in the adoption of the buttress and pilaster technology as part of a grass-roots process to empower local communities to construct more buildings that are earthquake-resistant (Blondet 2003).
Ring Beam
Another name for a ring beam is a seismic band. This technology works by tying walls together in a rectangle to increase their stress resistance. Load-bearing masonry construction widely adopts this technique by applying ring reams continuously (like a belt) (Blondet 2003). These ring beams support the roof (made using timber or concrete). Besides the ring beams, engineers also use truss-like timber on the lintel to support the ring beams and improve the efficiency of the buildings to withstand severe earthquakes (Blondet 2003).
Seismic Strengthening
Blondet (2003) outlines a detailed experiment aimed at establishing the best reinforcement methods for adobe houses. The experiment included the testing of wood, chicken wire mesh, and welded mesh. After undertaking a comprehensive analysis of these materials, he established that welded wire mesh was the best method for reinforcing adobe houses (Blondet 2003). Using bottle caps, the wire mesh hooks to the wall as outlined below
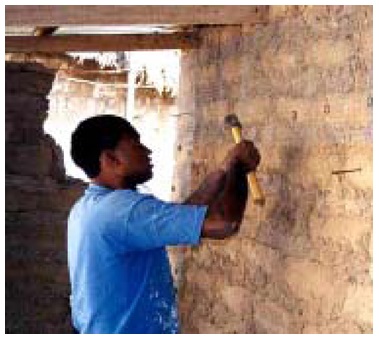
Placed in horizontal and vertical stripes, the wire mesh cement and sand mortar cover the mesh such that the building does not easily collapse if an earthquake occurs. The success of reinforced buildings manifested through the ability of reinforced buildings to withstand a past Peru earthquake. In the same earthquake, unreinforced adobe buildings suffered significant damage (Blondet 2003).
Protection of Historic Adobe Buildings
Even though some adobe buildings have a strong historic and cultural significance, they still suffer the same vulnerability as ordinary adobe buildings do. Thus, it is crucial to protect these buildings from severe damages so that their authenticity maintains (a careful emphasis to preserve buildings’ original fabric initiates these efforts). Several organizations (including the Getty Conservation Institute) have undertaken these efforts (Blondet 2003).
Dincel Wall
The use of polymer (as a new technology provided by the Dincel Construction System (DCS)) has shown tremendous success when used as a reinforcing building technology because when it mixes with concrete, it is easy to derive a “cost-effective, strong, durable and maintenance-free structure” (Dincel 2012, p. 3). This advantage not only highlights DCS’s capability to withstand harsh environments but also demonstrates the possible cost-saving advantages that occur from the use of the technology. For example, Dincel (2012) affirms that DCS buildings are usually maintenance-free. Besides this advantage, DCS technology is simple to use, versatile, and durable (Dincel 2012).
Conventional construction practices (especially in large-scale construction) require large cranes; however, DCS technology does not require this massive investment in construction tools because they are simple to build. Its simplicity saves time and costs associated with construction. Moreover, Dincel (2012) affirms, “DCS allows the construction of a building’s skeleton without interference from services and fit-out trades, with no formwork removal and reduced wet weather delays, while minimizing the risk of injury to workers” (p. 4). Swinburne University of technology confirms the above advantages by showing that apart from the energy and time savings associated with DCS technology; DCS also significantly reduces CO2 emissions (compared to traditional construction techniques) (Dincel 2012).
Since earthquakes may cause tsunamis and other disasters, the waterproof attribute associated with DCS technology also provides a guard against water destruction. This technology is especially crucial in seismic areas that are very close to large water bodies. For example, if Japan adopted this technology, it would have saved the need to buy new construction materials (destroyed by raging waters). DCS’s waterproof attribute explains its use in the construction of water tanks or other structures below the water table (Dincel 2012). This “waterproof” attribute complements the fact that DCS does not corrode (regardless of its exposure to seawater or petroleum).
One notable advantage regarding DCS technology (which also loudly resonates with the Japan earthquake disaster), is its insulation against fires. Many buildings and facilities burned in the Japan earthquake disaster because highly flammable materials characterized their construction. However, if manufacturers used DCS to construct the materials, the capability of the buildings to withstand fires would have significantly improved. Therefore, in the event that fire occurs (because of burst gas pipes or other reasons), the DCS technique provides a short-term measure to prevent fire destruction (before people employ other measures to arrest the situation).
The ability of DCS to withstand fire is lower than the threshold set by the Building Council of Australia. Dincel (2012) demonstrates that the advantage of using DCS technology is widespread because this material does not crack or deteriorate (excessively) from long exposures to fire and water. Its ability to withstand strong forces possibly highlights its greatest advantage to withstand earthquakes because Dincel (2012) demonstrates the ability of earthquakes to trigger a sideways load on buildings. DCS techniques withstand these forces.
Dincel construction System developed the Dincel wall to withstand earthquakes of up to magnitude nine on the Richter scale. An earthquake of magnitude nine is very strong because the strongest earthquake ever recorded was magnitude 9.5 (in Chile) (Dincel 2012). The University of Technology Sydney is one institution that has confirmed the effectiveness of the Dincel wall in minimising the impact of earthquakes. Compared to traditional construction measures of minimising the impact of earthquakes, the Dincel concept is relatively more effective.
From the higher threshold to withstand an external force, the Dincel method is relatively more effective than conventional approaches to mitigating earthquakes. Moreover, the superior ratios of 2:1 for accommodating large displacements demonstrate the superiority of the Dincel wall over other techniques. This effectiveness is especially useful in strengthening building structures if an earthquake occurs. One factor that makes the Dincel concept very effective is the reinforcement of concrete by a polymer skin. This advantage enables the concrete to withstand high levels of displacement. Through this advantage, buildings made from Dincel technology may withstand a displacement level of up to 4.4% (traditional construction techniques crumble when subjected to displacement levels of only 2.5%) (Dincel 2012).
Finally, it is crucial to point out that DCS technology gives buildings a 100-year lifespan. Furthermore, the technology is environmentally friendly because it uses 100% recyclable materials. Currently, more than 300 projects (within Australia alone) used DCS technology. More than 90 engineering companies have also recommended its use around the country. This high approval rate explains the reason for the attainment of the Innovation New Product Award, which DCS Company received in 2009 (Dincel 2012).
Finally, the ability of building materials to absorb seismic forces also characterizes their effectiveness in building earthquake-resistant buildings. Therefore, the capacity for a building to dampen some of this energy depends on the ductility of the materials used to construct it. Materials such as concrete or plastics have low ductility because they absorb little energy (when compared to ductile materials such as rubber). The use of materials with low ductility is potentially disastrous for buildings because even mild earthquakes may potentially cause the collapse of a building. However, buildings made of steel and concrete are less vulnerable to earthquakes because they have a higher ductility. Structural steel however offers the highest ductility because its design occurs in dynamic shapes and sizes (Harris 2012). These shapes sustain a high ductility because they enable buildings to bend without completely collapsing. The base-isolation technique is a feasible and effective strategy for developing earthquake-resistant buildings. Many parts of the world accept this technology including the U.S where the National Earthquake Hazards reduction program says more than 200 buildings in the country adopted this technology (most of these buildings are government and fire department buildings) (Harris 2012). The modifications to these buildings occurred after the 1989 Loma Prieta earthquake.
Improving Building Foundations
Harris (2012) says buildings can only be earthquake-resistant if their design accommodates sideways forces. He also emphasizes the importance of light buildings because he believes lighter buildings exert a lower force on the foundation (this is especially true when buildings have a higher load on the upper floors). Therefore, Harris (2012) recommends that roofs should strategically have a lighter material. Common knowledge dictates that when buildings stand on weak foundations, they have a higher chance of collapsing if an earthquake occurs (regardless of any advanced engineering technologies employed). However (assuming that a building has a strong foundation), it is easier for engineers to design a building’s foundation to withstand seismic forces (Harris 2012). Earthquakes make buildings collapse because it shakes their foundations. To minimize this effect, Harris (2012) encourages engineers to reinforce a building’s foundation in an interconnected manner (where the building’s foundation links with the building itself). Therefore, when an earthquake occurs and shakes a building’s foundation, it has to shake the entire building too (Harris 2012).
Base Isolation
Base isolation is one technology that minimizes the weaknesses of a building’s foundation to collapse because it floats a building above its foundation (to do so, a building uses a system of bearings, springs, and padded cylinders). The bearing links with the foundation of the building through plates of steel. Therefore, whenever there is an earthquake, the foundation of the building may move but its impact on the building reduces. This technique works by reducing the building’s horizontal motion to cause minimum impact on the building. Minimal damage, therefore, occurs on a building because regardless of the presence of the base isolation system, some degree of vibration still occurs on the building
The Research Foundation of the State of New York (2010) says the main principles informing advanced earthquake resistance technology is not to strengthen the buildings but rather, to reduce the impact of the earthquake on the building. Base isolation and energy dissipation technologies are among the most notable technologies for reducing an earthquake’s impact on a building.
The lead-rubber bearing is among the most commonly used base-isolation tools because it is stiff in the vertical direction but very flexible in the horizontal direction. The lead-rubber bearing technique characterizes the combination of rubber and steel to form a solid lead plug in the middle. These steel plates connect the building and the foundation (Research Foundation of the State of New York 2010). The following diagram shows a description of how the base-isolation system works.
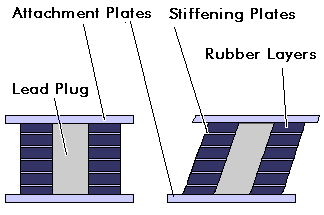
The diagram shows how the base isolation system counters seismic forces on a fixed-based building. When an earthquake starts, the building’s base-isolation system counters this force by moving the building in the opposite direction. For example, when an earthquake causes a building to move to the right, the base isolation system counters this movement by making the building move to the left (technically, seismologists say the building displaces to the left) (Research Foundation of the State of New York 2010). Part of the reason the building moves in the opposite direction is because of inertia force. The Research Foundation of the State of New York (2010) says inertia force is among the most important forces present in an earthquake. In addition, it is crucial to understand that inertia forces are usually proportional to the acceleration of a building’s ground motion. Furthermore, The Research Foundation of the State of New York (2010) stresses the importance of realizing buildings do not just move in one direction. Therefore, because of the unpredictable movement of buildings (in an earthquake), it is vital to understand the ability of buildings to move in varying directions.
The following diagram shows the difference between a base-isolated building and a fixed-base building.
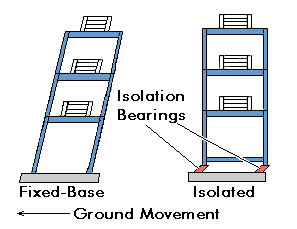
From the above diagram, the earthquake affects the fixed-base building and consequently, it loses its shape from a rectangle to a parallelogram (deformation). The base-isolated building however resists the inertia forces from the earthquake and retains its shape. The deformation of buildings in an earthquake occurs as the main reason buildings collapse under the forces of an earthquake. To understand further the impact of the base-isolation technology on buildings, it is vital to envision two pieces of wood held together at a right angle by some nails. When a rapid and unsettling force acts on the structure, the nails come out and the connection fails. This is what happens to fixed-based buildings.
Experiments aimed at testing the base-isolation system have proved that the technique can reduce up to one-quarter of the ground acceleration forces from earthquakes. This decrease also affects inertia forces because they are also dependent on the level of ground acceleration (Research Foundation of the State of New York 2010). The inertia force decreases because the base isolation system lengthens a building’s period of vibration because structures with a longer period of vibration have a better chance to resist earthquakes than structures that have a shorter period of vibration. The rubber isolation system (present in the base isolation system) also offers some cost advantages because the rubbers do not need replacement because of their high elasticity. However, the lead plug that holds the rubber together dissipates under the force of the earthquake and needs replacement (it generates a lot of energy as it dissipates, thereby reducing the energy that would be transmitted to the building). Through the reduction of energy, lead is able to dampen the building’s vibration thereby causing its eventual stability. Regarding this process, the Research Foundation of the State of New York (2010) says, “Damping is the fundamental property of all vibrating bodies which absorb the body’s energy of motion, and thus reduce the amplitude of vibrations until the body’s motion eventually ceases” (p. 12).
Spherical Sliding Isolation Systems
The use of lead and rubber highlight one type of technology used in the base isolation process. Spherical slide isolation systems also offer another mechanism for the isolation of a building’s base from its foundation. As the name suggests, the spherical slide system includes curved additions to the building’s support system to absorb any horizontal or vertical forces from earthquakes. In addition, by expanding or reducing the radius of curvature, it is similarly possible to increase or decrease the intensity of building vibrations (Constantinou 2007).
Many buildings around the world have incorporated the spherical sliding isolation system. The U.S court of appeal building in San Francisco is one such building. This building is a manifestation of the American renaissance style and is perhaps the best demonstration of the use of spherical sliding isolation systems in Western America. The building’s construction started in 1905 and it featured excellent architectural designs such as marble walls and statues (Constantinou 2007). By traditional building standards, the U.S Court of Appeal Building in San Francisco is enormous as it stands 25 meters above the street and weighs 57,000 metric tonnes (Constantinou 2007). The building has also experienced several earthquakes in the past (such as the 1906 San Francisco earthquake and the 1989 Loma Prieta earthquake). The building survived the 1906 earthquake but suffered significant damages in the 1989 earthquake. From this damage, the building’s interior walls suffered significant damages, which also reduced its seismic resistance. When these damages occurred, the building’s operations ended and engineers proposed the incorporation of seismic isolation systems to protect the building from future earthquakes. Engineers chose this process because it was the least intrusive on the building’s historical artefacts. Similarly, engineers chose this method because it had the least life cycle cost (Constantinou 2007).
Therefore, the cost and technical merit of the base isolation technique informed the decision of the engineers to adopt it as reinforcement to the court of Appeal building. Constantinou (2007) explains that “The technical reasons for the selection of the FPS system have been its extensive testing, its low profile, which allowed installation without cutting away part of the foundation’s wood pilings, and its unique construction, which minimised transmission of the overturning moment to the footings and averted additional reinforcement of the footings” (p. 4).
By the end of 1994, the building had a functional base isolation system.
Damping and Energy Dissipation
Damping and energy dissipation is also effective ways of improving a building’s resistance to earthquakes (lead rubber bearings offer most of the dissipation capability). As noted in this paper, earthquake motions transfer a lot of energy to buildings. Naturally, buildings have a small potential to dissipate this energy but their capability to do so does not prevent their collapse. Therefore, to dissipate energy, buildings often collapse or weaken at the columns or beams (leading to severe damage) (Research Foundation of the State of New York 2010). Consequently, by increasing a building’s capability to damp energy, it is easier to protect the building from severe damage by reducing the seismic force acting on it. Accordingly, engineers have designed and developed many energy dissipation inventions for reducing seismic energy. These inventions broadly group into four categories that include friction dampers, metallic dampers, visco-elastic dampers, and viscous dampers (Research Foundation of the State of New York 2010). Friction dampers use frictional energy to dissipate seismic energy, metallic dampers use deformation of metals to dissipate energy, visco-elastic dampers use controlled shearing of solids to dissipate energy and viscous dampers use forced movements to dissipate energy.
Visco-elastic Dampers
Visco-elastic dampers not only reduce seismic force against earthquakes but also against strong winds. Like other dampers, a visco-elastic damper works like a car’s shock absorber where little shock transmits to the car’s chassis. In the same regard, the visco-elastic damper works by transmitting little energy to a building. This technology works by incorporating viscoelastic materials to steel plates so that there is little movement realized when the steel plates move because of seismic ground forces (Research Foundation of the State of New York 2010). The visco-elastic dampers exhibit non-linear behavior when they experience high strain (but its difference with other forms of damping techniques rests in its ability to portray viscous and elastic behavior). A purely elastic material usually behaves by storing all energy (under strain) and releasing the same energy when the strain disappears. The opposite of an elastic material would be a viscous material, which does not release any stored energy (all energy is lost as pure damping). Sometimes, the stress may be equal to the strain but the degree of stress or strain determines the viscosity. Here, only damping occurs, as stiffness remains nonexistent.
Globally, many buildings use visco-elastic dampers but perhaps the best example to demonstrate the acceptability of this technique is its incorporation in the construction of the world trade center. The World Trade Centre uses the visco-elastic dampers as two layers of sheer dampers. Here, rubber derivatives glue to steel plates and angle irons so that when these materials experience intense strain, the rubber cushioning acts as a buffer to store this energy and release it later (Research Foundation of the State of New York 2010). Before using this technology, different laboratories around the world (including Taiwan, the U.S, and Japan) affirmed its efficacy
Friction Dampers
Like other damping technologies, friction dampers incorporate in steel bracing to reduce the impact of seismic ground forces. Broadly, like other dumping technologies, the friction damping technique significantly reduces earthquake resistance and damage control potential of a building because when an earthquake occurs, the friction dampers move to a predetermined load position (to dissipate seismic energy) (Research Foundation of the State of New York 2010). The friction dampers, therefore, offer support to the walls so that the ductility of the building materials is not the only buffer for insulating the building against seismic energy. Comprehensively, the structural elements of a building shield from intense seismic forces. This system is also beneficial because it offers several advantages.
India has used friction dampers to construct apartment blocks. For example, the La Gardenia Housing complex in India (New Delhi) incorporates friction-damping technology to build seven towers of 18 storeys (Research Foundation of the State of New York 2010). Besides improving the earthquake resistance of the Indian buildings, the use of friction dampers eliminated the need for relying on concrete shear walls and member ductility to absorb seismic force in an earthquake.
Metallic Dampers
Metallic dampers help to dissipate seismic energy. Buildings that have metallic dampers incorporate separate metallic dampers as a tool for absorbing seismic energy (similar to the way X-shaped and triangular-based dampers work). The use of metallic dampers in seismic engineering started after initial experiments showed promising results. Afterward, engineers started exploring the use of this technology on real structures. New Zealand stands out among the first countries to adopt metallic damper technology in its construction industry. Using the technology, New Zealand buildings improved their seismic response level, thereby significantly improving their earthquake resistance level. Research Foundation of the State of New York (2010) gives an example of the collapse of the six-story cardiology hospital complex in Mexico City, which failed to resist a severe earthquake that happened in 1985 (as a representation of the importance to incorporate metallic damper technology). After the earthquake, engineers fixed 18 metallic metal dampers on the building’s floor and the results showed an increased potential for the building to reduce its inter-story drift. This increased effectiveness occurred because of increased stiffness and increased energy dissipation. The metallic damper works by deforming under the pressure of seismic forces without deflection concentration (however, the metallic deformation may happen when the metallic dissipaters experience little force). The deformation under small disturbance happens because out-of-plane stiffness is often minimal (Research Foundation of the State of New York 2010).
Fluid Viscous Dampers
Fluid viscous dampers have very high applicability in aerospace dynamics and the development of defense systems. The application of fluid viscous dampers in seismic engineering only started in 1999 when two companies explored its use in seismic engineering (Constantinou 2011). Through a rigorous technological interchange, both organizations decided to engage in a similarly rigorous experiment to incorporate fluid viscous dampers as an augmentation of the base isolation system. To test the efficiency of fluid viscous dampers, engineers used steel-framed building models in the experiment and all of them showed high resistance to heavy seismic loads. For example, the experiment showed that a three-story building, which lacked these fluid viscous dampers, was on the verge of yielding when it experienced a third of the force experienced in the 1940 El Centro earthquake (Constantinou 2011). With bolts added to six fluid viscous dampers, the experiment showed that the use of fluid viscous dampers in seismic engineering could improve a building’s ability to sustain earthquakes, which were more than 150% the force experienced in the 1940 El Centro earthquake as well (Constantinou 2011). The experiment showed that the building withstood the earthquake without any significant damage because the inclusion of fluid viscous dampers increased the building’s seismic strength by more than three times. The fluid viscous design used in the above experiment was closely similar to the design used in the B2 stealth bomber. Successful experiments used on reinforced building models are similar to those used in the US Navy Tomahawk cruise missile (because of the history of fluid viscous technology in the military).
Fluid viscous dampers work by transmitting high-velocity fluids through orifices (to buildings) to prevent them from experiencing significant damage if an earthquake occurs. The above experiments affirmed that fluid viscous dampers were effective in reducing drifts and maintaining shear forces at controllable levels (or less than the forces experienced when a building lacked fluid viscous dampers). The main drawback of this method is its visco-elastic nature, which introduces a substantially axial force component that is out-of-phase with drifts and column base moments (Constantinou 2011). Engineers tested these attributes on shake tables (with two and three-story building model structures). “The experimental results demonstrated reductions of drifts and shear forces of the order of two to three in comparison to the response of the models without dampers for a wide range of earthquake input motions” (Constantinou 2011, p. 9). This paper also demonstrates that fluid-damping technology also integrates with base isolation technology to enhance a building’s energy dissipation capability. Tests have also been undertaken using this addition and the results showed that simultaneous isolation bearing techniques were more effective when compared to structures that did not have the fluid displacement addition.
Through this experiment, several buildings have incorporated this technology in their design process. One such building is the San Bernadino County Medical Centre, which comprises five structures (Constantinou 2011). Cumulatively, these buildings use 233 fluid viscous dampers with an output of 320,000 lbs each (Constantinou 2011). In addition, these fluid dampers have a displacement of 48 inches each. Constantinou (2011) says, each viscous damper
“generates an energy dissipation level of 3,000 horsepower at a speed of 60 inches per second. The 60 inches per second speed compares to the 51 inches per second maximum peak ground velocity for the damaging Northridge earthquake of 1994 and shows the tremendous seismic capacity desired for the medical center” (p. 9).
Future Technologies
The main goal of designing an earthquake-resistant building is to protect human life and property. Harris (2012) says that if a building’s design allows people enough time to escape (if an earthquake occurs), it should be regarded as an earthquake-resistant building. Greg Deierlein (from Stanford) has interpreted the future of earthquake-resistant buildings as the ability to repair deformed buildings after an earthquake (Harris 2012). Jerome Hajjar (from North-eastern University) also shares this view because he says the future of seismic engineering is the ability to return buildings back to their original forms after an earthquake (Harris 2012). These two scholars are working on a new technology called the rocking frame, which incorporates steel frames, steel cables, and steel fuses to reinforce buildings against an earthquake. The technology works by using steel frames to direct seismic energy to gnash fuses that prevent a building from collapsing. Once the earthquake ends, the building takes its initial posture and the replacement of destroyed fuses occurs. The objective of this technology is to fix the building quickly and resume occupancy immediately.
Another innovation that is under exploration is the seismic invisibility cloak. This technology aims to make buildings transparent to the forces of an earthquake. To achieve this objective, the pioneers of these technologies propose the compression of many concentric containers on a building’s foundation. Therefore, when seismic waves try to shake a building, they compress into a bottleneck. To explain how the technology works, Harris (2012) says, “the waves zip by, just beneath the building’s foundation, and exit the rings on the other side, where they resume their original speed and amplitude” (p. 5).
Interestingly, Harris (2012) also says that the future of seismic engineering should not look ahead but look into the past. He makes this statement by highlighting the importance of devising new waves to make earthquake-resistant buildings the best way to circumnavigate the problem of property destruction during earthquakes (because it is more expensive to build new structures than maintain old ones). Nonetheless, any innovative engineering practice for resisting earthquakes remains a prototype until it experiences real earthquakes.
Seismic Testing
The largest seismic testing for the above finding is the capstone experiment, which occurred in Japan (between June and July 2009). The test involved Colorado state University (the project leader) and Simpson Strong-tie (Colorado’s partner) (Pryor 2010). The experiment subjected a seven-storey building to simulated seismic forces in E-defence laboratories. The seven-storey building is comprised of a ground retail area made of steel and six storeys of residential floors made of wood. The use of the steel (to construct the first floor) occurred after incorporating the latest technology in beam-column protection. In addition, “the entire structure was designed using a new direct displacement design methodology weaved into a performance-based seismic design framework” (Pryor 2010, p. 1). Comprehensively, the experiment showed that when wood and steel are carefully used together to build a mid-rise building, the level of earthquake resistance significantly improves.
Credibility of the Experiment
The credibility of this experiment comes into sharp focus because through the validity of the experiment, it is easier to rely on and replicate the findings across other spheres of construction. One factor that complements the validity of the outcome is the occurrence of the experiment in an ultramodern environment (E-defence facility). This facility is the largest in Japan and its development started after the Kobe earthquake. Its capability to test a structural facility is high because structural products stand on a shake table that is able to move in three directions at the same time (Pryor 2010). This multiplicity emulates the real-life potential of earthquakes (to move in three directions). Furthermore, “with surface dimensions of about 50 x 65 feet, the table can support test buildings weighing up to 2.5 million pounds and has a range of motion in excess of six feet in all directions” (Pryor 2010, p. 7). The capstone experiment used most of the available space because its measurements almost filled the entire test table.
The use of wooden structures in the capstone experiment mirrors the increased use of wood as the main building material in active seismic areas. More so, with the increased adoption of green building concepts, wood has become very popular building material for commercial and residential development. While wood has demonstrated a good performance during earthquakes and similar events, the increased adoption of wood as the main building material has fundamentally changed the nature of mid-size buildings over the past five decades. Therefore, with the increased adoption of engineering lumber methods in wood construction, buildings have less redundancy and lateral strength. Moreover, the increased use of engineering lumber methods has seen a drastic reduction in interior walls and the expansion of roofs and floors. However, there has been an increased demand for more openings on the remaining walls and the subsequent concentration of lateral demand into shorter wall segments (from the understanding of the true system-level behavior of similar structures).
In the past decade, few scientists have bothered to undertake a shake-table test for wooden structures. The capstone experiment is therefore unique in this regard because the scale of the experiment predicts the behaviour of mid-size buildings. Other experiments, which have occurred in the past, cannot predict the behaviour of a bigger building magnitude because they use smaller buildings in their experiments. For example, “The 2000 CUREE-Caltech Wood frame Project tested a modest two-story structure under uniaxial ground motion and was an important step in understanding nonlinear modeling issues as well as the influence of non-structural finish materials” (Pryor 2010, p. 8). The CUREE-Caltech Wood frame Project also involved a similar study (to the capstone experiment), which involved underground parking. The test showed buildings with an underground parking system were more vulnerable to earthquakes and could easily collapse. An earlier experiment tested a larger 1,800 square foot house of two storeys on a shaking table and confirmed the performance of such a building on a California-type earthquake (according to California building code standards) (International Association for Earthquake Engineering 2002). Here, the engineers used the 1988 Uniform building code. This test not only predicted the performance of buildings according to the 1988 Uniform Building code but also provided another set of measurements to ascertain the success of predictive modeling (Pryor 2010). Therefore, the test provided a platform for augmenting previous findings with current building standards (this comparison occurred with respect to non-structural finish materials). The non-structural building materials added strength, stiffness, and damping – these measures are widely used in today’s building standards.
The above experiments have revolutionized the understanding of seismic activities on mid-size buildings and paved the way for the adoption of performance-based seismic designs. Their methodologies emphasize the reliability of structural modeling as a correct predictor of a building’s performance (in an earthquake). However, (Pryor 2010) cautions that it is difficult for wooden structures to perform according to predictions because the load path is not accurate like buildings made of concrete or steel. Nonetheless, Pryor (2010) says,
“The Capstone project, given the large size of the test structure and triaxial input motions, represents a quantum leap forward in providing data to test and refine researchers’ abilities to predict structural response as well as validate new and existing construction methods” (p. 9).
Furthermore, to have an accurate assessment of the experiment’s findings, more than 200 sensors collected data. For example, the optical tracking method was used to record data about the external environment, while strain gauges established tie-down forces and floor accelerations. Lastly, string potentiometers measured the level of deformation and the significant movements realized from the structure’s response to ground accelerations (Pryor 2010). Through these measurement tools, the drifts measured did not exceed 1.25%. In fact, the last shake was the strongest and the strongest drift ever recorded was less than 3% (Pryor 2010). Damage occurred from ground accelerations but none of it was structural (only small cracks on the wall occurred). The engineers, therefore, agreed that the results indicated very good performance. This performance also indicated that the direct displacement design was beneficial and reliable. Pryor (2010) however observes that one area of high interest that intrigued him was the measurement of tie-down forces. He highlights the inconsistent response of shear wall response between segmented and perforated walls as one area of key interest (Pryor 2010). The closeness of the predicted and measured demands was also very similar to the measurements derived from anticipated tie-down forces because Pryor (2010) claims, “130 kips was measured in the lowest rods in a six-story tall shear wall stack comprised of typical double and single-sided wood structural panel sheathed shear wall construction” (p. 9).
From the findings of this experiment, several national building authorities have encouraged the use of wooden multi-storeyed structured. For example, the government of British Columbia in Canada allows the use of light-frame wood construction for up to six storeys because of its short construction cycle and reduced cost of construction. This trend has increased in several other parts of the world because the capstone project has proved it effective to use light-frame construction techniques in seismic regions.
California State Office building designed building after undertaking an acceptable testing program, which incorporated the facilities of the University of Buffalo and Taylor. Comprehensively, engineers established that a building made of steel or concrete could increase its seismic strength by using fluid viscous damping technology. This addition to seismic engineering shows the transition of useful technologies of the cold war era to the current efforts aimed at protecting human life through reliable building and construction practices.
Safety Design and Policy Implementations
Liquefied Natural Storage Gas in Greece
Liquefied Natural Gas (LNG) is a dangerous commodity because it is highly inflammable. Therefore, the mode and design of its storage (in seismic areas) needs to occur in a cautious manner because of the potential dangers that may arise when an earthquake occurs. Therefore, managers need to develop plant operations and safety aspects of LNG storage with the highest emphasis on safety (Research Foundation of the State of New York 2010, p. 9).
The designs of LNG storage tanks have a lot of seismic significance because hydrostatic stresses are tested and prevented at the design stage. However, it is crucial to point out that the testing process normally involves the use of water instead of LNG because the latter only has a unit of weight of just less than that of water. Once the storage tanks fill with water, a higher combined hydrostatic stress occurs when moderate seismic excitation occurs. Beyond a certain degree, seismic loading dominates the main loading condition (Greece experiences this loading). Instead of modifying the shape, size, or geometry of the storage tank, a less drastic but effective technique for reducing the seismic force is adopted – base isolation.
This technique has been widely adopted in Greece. Two storage tanks under construction in Revithoussa Island are set to incorporate this modification. The bearing design (which also incorporates the tank’s design) closely resembles those adopted in the US court building in San Francisco.
Japanese Nuclear Plants
Nuclear plants pose a significant safety risk for humans because of the dangers posed by radioactive leaks. Several factors may cause radioactive leaks but earthquakes are dangerous to nuclear power plants because they destabilize nuclear facilities. Since nuclear power plants are close to large water bodies, about 20% of the world’s nuclear power plants suffer the risk of damage because of seismic activity (World Nuclear Association 2012). Many countries have taken drastic measures to ensure their plants are able to withstand the most drastic earthquakes. For example, nuclear power plants in France withstand very strong earthquakes such as the 1000-year calculated event for each site. Globally, the International Atomic Energy Agency (IAEA) reviews the seismic risks for nuclear power plants and the dangers posed by these plants if an earthquake occurs (World Nuclear Association 2012).
Since Japan sits on a high-risk seismic activity area, engineers are attentive to the design, sitting, and construction of nuclear power plants. Designers and constructors use many methods to prevent nuclear disasters, but the most common way is the installation of seismic detectors (International Association for Earthquake Engineering 2003). The seismic indicators work by detecting significant seismic activity and shutting down the plants whenever an earthquake reaches a specific threshold. The threshold for seismic activity was set at 135 Gal in Fukushima nuclear plant (World Nuclear Association 2012). The logarithmic Richter magnitude scale often measures the energy released in an earthquake, but unfortunately, this measure does not often mirror the forces produced by the earthquake. For example, World Nuclear Association (2012) says,
“Japan has a seismic intensity scale in shindo units zero to seven, with weak/strong divisions at the fifth and sixth levels, hence ten levels. This describes the surface intensity at particular places instead of the magnitude of the earthquake itself” (p. 3).
Recently, the Japanese government reviewed its seismic design plans to be sensitive to an earthquake of about 6.7 (before this review, Japan’s seismic design indicated that an earthquake of S1 was the most severe earthquake anticipated in a nuclear power plant) (World Nuclear Association 2012). However, the sensors could act to lower levels of earthquake intensity. The revised measure for earthquake sensitivity was only released in 2007 and it was designed to react to an earthquake of 6.7 magnitudes (initially, it was designed to react to an earthquake of 6.5 magnitudes) (World Nuclear Association 2012).
The 7.2 magnitude Kobe earthquake that hit Japan in 1995 initiated the above review of Japan’s nuclear power design guidelines because media reports highlighted the vulnerability of the surrounding nuclear plants to similar earthquakes. In the Kobe earthquake, observers reported a horizontal ground acceleration of 817 Gal and a vertical ground acceleration of 332 Gal (World Nuclear Association 2012). Both measures were above the anticipated levels. Fortunately, based on the earthquake-proof measures installed on the affected nuclear power plants, no nuclear power plant (within a 200-kilometer radius of the earthquake) suffered any functional damages. These power plants include Takahama and Oki power plants (which is located only 130 kilometers from the epicenter of the earthquake), the Mihama power plant (which is located only 180 kilometers away from the epicenter of the earthquake), and research reactors in Osaka and Kyoto. The power plants, which were operational at the time also continued to operate at maximum capacity. Through the same disaster, the Japanese government set up new building and road construction standards to withstand an earthquake of 7.75 magnitudes (the Kobe earthquake was only 7.2) (World Nuclear Association 2012). The ineffectiveness of old design guidelines, which failed to predict damage to structures inform the review of seismic design and construction guidelines. For example, engineers consider Peak Ground Acceleration (PGA) to be an ineffective way for predicting structural damage to nuclear facilities and seismologists are proposing the adoption of Cumulative Average Velocity (CAV) in its place.
An earthquake of 7.3 magnitudes once hit Japan in the year 2000 and forced Japanese geological experts to warrant a review of prevailing seismic guidelines because the damage realized from the earthquake exceeded the expectations of the experts (the adoption of old seismic design guidelines occurred in the eighties and were minimally reviewed in 2001). The 2000 earthquake forced the Japanese government to publish a new set of guidelines in 2006 (World Nuclear Association 2012). The result of this review was the replacement of the S1-S2 guidelines for a more effective Design Basis Earthquake Ground Motion criterion that uses Gal measurements. Initially, the adoption of new seismic design standards was poor but after a series of earthquakes (including magnitude 9.0 Tohoku-Taiheiyou-Oki earthquake and the 2007 Kashiwazaki Kariwa earthquake), nuclear facilities upgraded their design estimates (World Nuclear Association 2012).
Several Japanese nuclear power plants are located in seismic areas and no nuclear power plant bears a high seismic risk as to the Hamaoka nuclear power plant (located near Tokai). In fact, after reviewing the history of Tokai, several earthquakes in the past century and a half have happened in the region. Since the last bug earthquake happened, 155 years have passed (World Nuclear Association 2012). Because of the high-risk location of the Hamaoka nuclear power plant, the plant’s designers constructed the building to be sensitive to an earthquake of S1 of 450 Gal and S2 of 600 Gal (recently the upgrade of several units occurred to attain a new standard of 1000 Gal). The effectiveness of these measures manifested in 2009 when the nuclear plant automatically shut down after the detection of a 6.5 magnitude earthquake (World Nuclear Association 2012). Two units of the same plant also previously closed because of the lack of seismic upgrading (after evaluating the cost of upgrading the facilities, it was thought that it would be cheaper to build new plant units). The same efficiency manifested in the US when a nuclear plant (North Anna) automatically shut down in 2011 after a 5.8 magnitude was recorded (World Nuclear Association 2012). This earthquake produced 255 Gal of ground acceleration but the plant could respond to only 176 Gal. Eventually, the plant automatically shut down and no significant damages occurred (World Nuclear Association 2012). After a thorough safety evaluation review, the plant was later operational.
Another success story is the 1999 Taiwan earthquake where three nuclear reactors in the Northern part of the country completely shut down after detecting seismic activities (thereby preventing any functional damages or severe accidents) (World Nuclear Association 2012). The earthquake had a 7.6 magnitude and caused the loss of thousands of human lives. However, after only two days after shutting down, the nuclear plants restarted and operations normalized (minimal damage, however, occurred on one nuclear reactor distribution line but the rest of the plants resumed full operations). Through the effective seismic designs incorporated in the design of the nuclear power plants, power was quickly restored to the industry.
In 2005, a 7.2 magnitude earthquake hit Japan. This earthquake mainly affected Northeast Honshu where three nuclear power plants were located. However, no damage occurred from these nuclear power plants because they immediately shut down before any damages occurred. According to the S1 design basis, the nuclear power plants could trip after sensing only 200 Gal of earth movements. According to the S2 design, the power plants could also trip at 350 Gal. Onagawa (the main power plant affected) later restarted in 2006 after seismic reviews showed it could withstand an earthquake of 8.2 (World Nuclear Association 2012).
In June 2006, another earthquake of magnitude 6.8 hit the coast of Japan. This earthquake was bound to disrupt the operations of the Kashiwazaki Kariwa nuclear power plant, which was located only 16 kilometers from the epicenter of the earthquake. The geological environment of the region however magnified the seismic intensity of the plant because the World Nuclear Association (2012) says, the peak ground acceleration from the earthquake worsened because of the geological nature of the environment. The S1 and S2 values of the plant therefore exceeded and the plant shut down promptly. The shutdown included the stoppage of four operators. Three operators were also not working because they were idle when the earthquake struck (World Nuclear Association 2012, p. 15).
Nonetheless, there were many incidences reported from the earthquake but none of them posed a significant barrier to the operations of the plant. The safety of the plant, therefore, was sustained (despite the intensity of the earthquake producing intense ground accelerations, which were almost three times the anticipated strength). Once the International Nuclear Event Scale investigated the plant, they said the damage realized on the nuclear plant was insignificant and did not compromise the safety of the plant (World Nuclear Association 2012). However, albeit no significant damage occurred from this earthquake, safety agencies recommended the adoption of more earthquake-resistant mechanisms (World Nuclear Association 2012). Comprehensively, from the above operations, nuclear power plants have benefitted from improved safety mechanisms of earthquake-resistant technologies. Furthermore, the resilience of these plants (in the wake of severe earthquakes) shows that policy guidelines regarding the design and construction of nuclear power plants can be effective in ensuring designers and contractors develop earthquake-resistant buildings/facilities (World Nuclear Association 2012).
Recommendations and Conclusion
From the pieces of evidence gathered in this paper, earthquakes have caused immense human and property loss. Besides the valuable human lives lost, earthquakes have caused monetary losses rising to billions of dollars. The Recent Japan earthquake and its subsequent threats to the global ecosystem is just one example of this devastation. The sheer magnitude of earthquakes and their capability to cause other natural disasters (like tsunamis) also underscore their threat to humanity. Moreover, the threat of these natural disasters on human activities such as farming and nuclear power generation poses new challenges to the survival of the human species and modern societies. However, these natural activities pile more pressure on seismic engineers to reduce their impact on society so that people can rebuild their lives even after these disasters.
In the wake of this need, engineers have tried to design and construct earthquake-resistant buildings. Their commitment however lies in their conviction to construct earthquake-proof buildings that are able to withstand even the most severe earthquakes. Engineers, therefore, need to provide the surety that earthquake-proof buildings can look as good as new once the shaking ends (International Association for Earthquake Engineering 2002). earthquake-proof buildings should adopt new technologies and processes in detecting and reducing earthquake forces.
This paper identifies the base isolation systems, reinforcement of building materials, proper choice of building materials, and effective formulation and implementation of safety guidelines as the main pillars underlying the construction of earthquake-resistant buildings. The adoption of earthquake-resistant technology in a seismic area supports the adoption of this technique. The findings of this paper are therefore useful in improving the ability of buildings to withstand seismic forces.
This paper highlights the importance of adopting earthquake-resistant methods when reducing a building’s vulnerability to seismic forces. This paper also demonstrates the influence of earthquakes on buildings by highlighting their potential to weaken their foundation and ability to sustain lateral loads. To mitigate these areas of vulnerability, this paper establishes that using less dangerous materials such as wood and reinforcing steel and concrete improves a building’s ductility and ability to withstand seismic forces. This paper also demonstrates the importance of adopting base-isolation mechanisms as a reliable way to insulate buildings from seismic forces. So far, this study proposes the base-isolation method as a cost-effective and sustainable method of construction. Besides the above techniques, implementing and adhering to high safety standards in the construction and maintenance of buildings also highlights one-way buildings may effectively pre-empt earthquake disasters. The establishment of seismic activity detectors has demonstrated high effectiveness in preventing nuclear disasters in Japan. The installation of these detectors occurred after adhering to high safety and design standards.
Many technologies explaining how to develop earthquake-resistant buildings stem from technical modifications. However, there are minimal non-technical advancements explored by researchers to achieve the same purpose. A few regulatory frameworks in the operations of nuclear plants surface as practical approaches for reducing seismic activity. The immense potential, therefore, exists in identifying preventive initiatives that similarly reduce the impact of earthquakes on buildings. The measures identified in this study, therefore, work to mitigate the impact of an earthquake when it occurs. More research should therefore occur to identify preventive measures that mitigate the impact of earthquakes on buildings (and especially on the people that reside in them). Such measures may involve the installation of seismic activity detectors and the like.
Finally, perhaps the biggest mistake people make when earthquake disasters occur is to forget its effects. The International Association for Earthquake Engineering (2002) says this is perfect human nature but within the engineering circle, professionals always come together and discuss ways that prevent future catastrophes. From these discussions, several alternatives manifest to alter traditional construction practices and make them more resistant to earthquakes. However, because of the false sense of security people have after they have forgotten the devastating effects of an earthquake, the momentum to change building structures and make them more earthquake-resistant is lost. In fact, the International Association for Earthquake Engineering (2002) says, it requires another earthquake to remind people of the need for engineers to devise newer techniques to strengthen buildings.
References
Agarwal, P 2006, earthquake-resistant Design of Structures. PHI Learning Pvt. Ltd., New York.
Amidon, M 2011, 2011 Japan Disasters, ABDO, New York.
Blondet, M 2003, Earthquake-Resistant Construction of Adobe Buildings: A Tutorial. Earthquake Engineering Research Institute, Oakland, California.
Cardenas, L 2012, The Chilean Earthquake and Tsunami 2010: A Multidisciplinary Study of Mw8.8, Maule, WIT Press, New York.
Constantinou, M 2007, Protective Systems for Buildings: Application of Spherical Sliding Isolation Systems, Web.
Constantinou, M 2011, Application of Fluid Viscous Dampers to Earthquake Resistant Design, Web.
Denny, M 2010, Super Structures: The Science of Bridges, Buildings, Dams, and Other Feats of Engineering, JHU Press, New York.
Dincel, B 2012 Dincel Construction Systems, Web.
Ford, E 2012, Frommer’s Washington, D.C., John Wiley & Sons, New York.
Gupta, H 2011, Encyclopedia of Solid Earth Geophysics, Springer, New York.
Harris, W 2012, How Earthquake-resistant Buildings Work, Web.
International Association for Earthquake Engineering 2002, International handbook of earthquake and engineering seismology, Academic Press, London.
International Association for Earthquake Engineering 2003, International Handbook of Earthquake & Engineering Seismology, Academic Press, London.
Macdougall, J 2011, Why Geology Matters: Decoding the Past, Anticipating the Future, University of California Press, Los Angeles.
National Research Council (U.S.) 1985, Liquefaction of soils during earthquakes, National Academies, Washington.
O’Rourke, T 1992, The Loma Prieta, California, Earthquake of October 17, 1989: Marina District, DIANE Publishing, New York.
Pryor, S 2010, Seismic Testing, Web.
Research Foundation of the State of New York 2010, Advanced Earthquake Resistant Design Techniques, Web.
Rouhani, S 1996, Geostatistics for Environmental and Geotechnical Applications, ASTM International, New York.
SEAONC 2005, 1989 Loma Prieta Quake, Web.
Schiff, A 1995, Northridge Earthquake: Lifeline Performance and Post-Earthquake Response, ASCE Publications, New York.
Singh, A 1999, Implementation of Safety & Health on Construction Sites, Taylor & Francis, New York.
Storgaard, N 2010, Earthquakes and their effects on buildings, Web.
UCSD 2012, Earthquake Hazards, Web.
World Nuclear Association 2012, Nuclear Power Plants and Earthquakes, Web.